The views expressed in this paper are those of the writer(s) and are not necessarily those of the ARJ Editor or Answers in Genesis.
Abstract
Chicxulub, Mexico has become synonymous with the dinosaur extinction in the secular literature. It is often referred to as the “smoking gun.” But how much of the data really support an asteroid impact? This paper reviews the history of the Chicxulub site and critically examines all pertinent data. It addresses the findings of the most recent well drilling activity, the earlier oil wells at the site, and all relevant geophysical data. Many surprising results are discussed, including the less than expected amounts of diagnostic high pressure minerals, the limited amounts of pseudotachylyte and iridium and even the lack of significant melt-rich rock. Alternative explanations are offered to explain the shocked quartz, ring faults and the claimed “peak ring.” The ambiguous nature of the gravity anomaly is addressed and an alternative interpretation is offered. Conclusions are made that indicate the evidence for a large impact at Chicxulub may not be as strong as generally believed. A case can also be made that there was no impact. Although some meteorites likely struck the earth during the Flood, creation scientists need to critically assess each site before incorporating them into their Flood models.
Keywords: asteroid impact, meteorite impact, shocked quartz, peak ring, dinosaur extinction, tsunamis, Paleogene, Cretaceous, Tertiary, K-T, K-Pg, Chicxulub crater, iridium, pseudotachylyte, gravity anomaly
Introduction
One of the greatest secular mysteries of all time concerns the extinction of dinosaurs. This extinction is commonly called the K-T or K-Pg event because it occurs in rocks near the Cretaceous-Tertiary (now called the Paleogene or Pg) system boundary. The secular scientific consensus on the absolute date of the K-Pg extinction event used to be 65 million years before present (Ma), however this “absolute” date has changed more recently to closer to 66 Ma (Cohen et al 2013; Kuiper et al 2008). Although there have been well over 90 secular, published ideas attempting to explain this extinction, from dinosaur medical problems, overspecialization, competition with mammals, plant changes, climate changes, sea level changes to increased volcanism, the most popular remains an extraterrestrial impact (Charig 1993).
Extinction in the rock record can be defined as the highest and last stratigraphic occurrence of an organism as a fossil. In the global Flood model, layers of animal and plant communities were rapidly piled on top of one another, from various directions and in a seemingly global order, according to the tectonic activity taking place at that moment. Viewed in this manner, these last occurrences are not extinctions at all, in terms of the secular definition. Instead, they are merely the last record of various organisms trapped by the Flood waters. Some creation scientists think the K-Pg was about the high point of the Flood waters when all land animals finally succumbed and drowned (Snelling 2014). That may be why it is at this point that so many organisms seemed to simultaneously go extinct. It was most likely a major juncture in the Flood record.
Creation scientists Oard (2009, 2012, 2013, 2014) and Spencer (1998a, 1998b, 2013, 2014, 2015) have extensively discussed the possible bombardment of earth with extraterrestrial impacts. Spencer (2015) has most recently made the claim that the vast majority of the impacts likely occurred during Day 4 of Creation Week, and that a second bombardment, involving fewer impacts, possibly occurred during the Flood event. Oard has taken the more extreme view, suggesting that upwards of a few thousand impacts may have stuck the earth, mostly associated with the onset of the Flood (Oard 2014).
Although there are about 190 identified impacts listed in the Earth Impact Database (http://www.passc.net/EarthImpactDatabase/New%20website_05-2018/Index.html), many of these impacts have limited physical evidence to support them. Oard (2013) has pushed this even further, adding nearly all circular-shaped geologic features as likely impacts, including most of the sedimentary basins found on continents across the globe. He used the circular or semi-circular shapes of these folded rocks as his main guide in his interpretation (Oard 2013). However, he failed to present any physical evidence in support of his interpretations, leaving most of his “impacts” speculative and unverifiable.
Finally, the belief in the global effects of the Chicxulub impact has become so extreme that a group of scientists have claimed a tie exists between the impact in Mexico and the Deccan volcanism in India (Richards et al. 2015). They proposed that seismic waves from the impact directly affected the volcanic style and eruptive rates of the Deccan volcanism over 13,000 km away.
In light of the role impacts may or may not have played in a global cataclysmic event like the Flood, a review of the evidence at the Chicxulub site is in order. This paper begins with a review of the criteria used in identifying an impact and addresses the available and historical evidence surrounding the Chicxulub site. It continues with an examination of the 1950s and 1960s PEMEX oil well drilling data and the nature of the geophysical “crater” anomaly, assessing the findings from the recent drill cores collected by the International Continental Scientific Drilling Program (ICDP). Conclusions are made that indicate the evidence for a large impact at Chicxulub may not be as strong as generally believed.
Criteria for Identifying an Impact Site
French and Koeberl (2010) have put together a comprehensive list of criteria to assist with the identification of terrestrial impacts, splitting their list into diagnostic indicators and non-diagnostic features. However, they remind us that “Only the presence of diagnostic shock-metamorphic effects and, in some cases, the discovery of meteorites, or traces thereof, is generally accepted as unambiguous evidence for an impact origin” (French and Koeberl 2010, 123).
Most diagnostic criteria are found in the target rocks as few impacts leave traces of the actual impactor (French and Koeberl 2010). Accordingly, most diagnostic indicators are some type of evidence of high metamorphic conditions due to the shock waves generated by the impact. These include: the presence of chemical signatures like iridium, shatter cones, high-pressure glasses (diplectic), high-pressure minerals, high-temperature glasses and melts, and planar fractures (PFs) and planar deformation features (PDFs) in quartz (French and Koeberl 2010).
French and Koeberl (2010) also remind us that most geological features of an impact are not unique, including circular patterns of faults and fractures and even circular gravity anomalies. They explain that many of these features can be the result of more conventional geologic processes like volcanism or tectonic activity. As a result, their list of nondiagnostic features includes: the identification of circular morphology, circular structural deformation, circular geophysical anomalies, fracturing and brecciation, kink banding in micas, mosaicism in crystals, the presence of pseudotachylyte, igneous rocks and glasses, and spherules and microspherules (French and Koeberl 2010).
For these reasons, Grieve and Pilkington (1996, 404) have stated that “The burden of proof for an impact generally lies with the documentation of the occurrence of shock-metamorphic effects.” But as will be shown, even indicators of shock-metamorphism may not be as diagnostic as claimed.
Overview of the Chicxulub Site
The Chicxulub site was primarily identified from a circular gravity anomaly near the northwestern edge of the Yucatán Peninsula, Mexico (Penfield and Camargo 1981) (Fig. 1). Hildebrand et al. (1991) first interpreted the large, nearly circular-shaped gravity anomaly as an impact which they termed the Chicxulub Crater (Fig 2). The feature is approximately 180 km in diameter, allowing room for a 10 km wide asteroid or meteorite to have created the dimensions of the crater. There is however, disagreement on the exact size of the crater (Morgan et al. 1997).
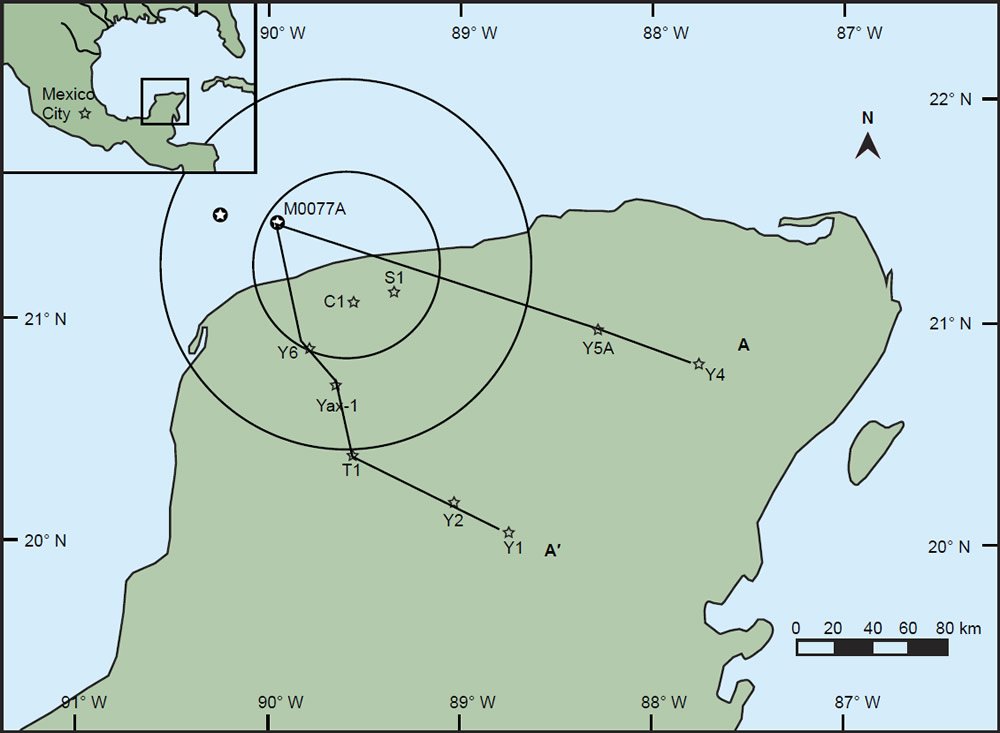
Fig. 1. Location map of the Chicxulub site, showing the PEMEX well locations and the two more recent boreholes M0077A and YAX-1. Modified from Tuchscherer et al. (2004). Circles show the postulated crater diameter at 100 km and 195 km distances. Figure courtesy of Susan Windsor.
The crater is not visible at the surface because it is covered over by younger, relatively undeformed Neogene sediments (Gilli et al. 2009). Therefore, it is generally assumed that the deeper rocks at the site (Cretaceous system and older) are the source of the circular gravity signature (Fig. 2). Several PEMEX Oil wells drilled into the crater have recovered cores of fused melt and broken and brecciated rocks in the pre-Paleogene section, confirming the likelihood of an impact (Fig. 1).
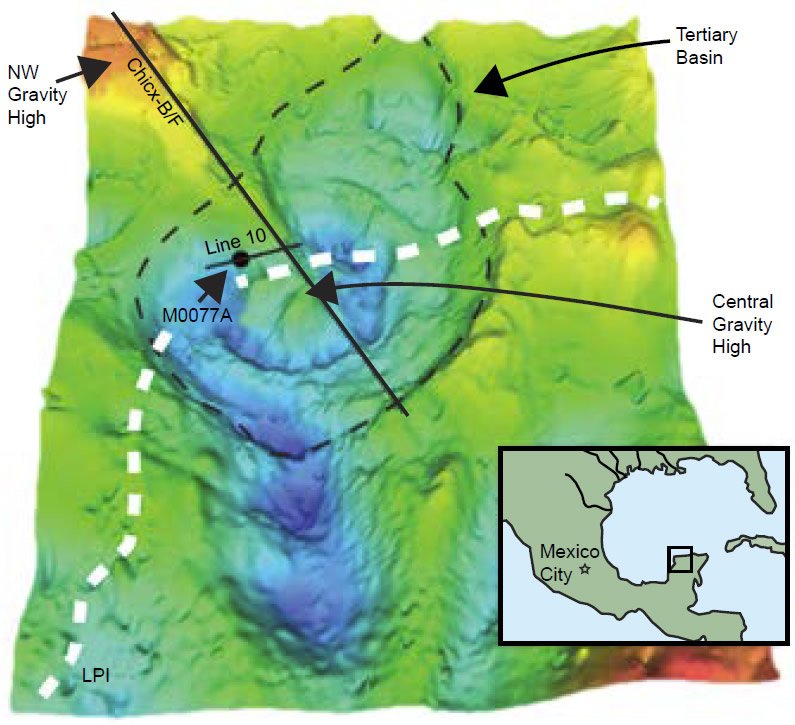
Fig. 2. Bouguer gravity map of the Chicxulub area, showing locations of features discussed in text, used by permission of Virgil Sharpton/LPI (Lunar and Planetary Institute). Location for seismic reflection Line 10 (Fig. 5) and wellbore M0077A are shown. High gravity anomalies shown in yellow and orange. Low gravity anomalies shown in blue. The white dashed line is the approximate location of the land/sea boundary. Figure courtesy of Susan Windsor.
The Evidence at Chicxulub
Sharpton et al (1993) reprocessed the gravity data over the Chicxulub site, finding the anomaly matched a three or possibly a four-ring impact basin (Fig. 2). These authors found gravity values in the Chicxulub basin to be about 20–30 mgal lower than regional values. However, in the center of the crater, they found a gravity high with values 15–20 mgal greater than the surrounding basin.
Sharpton et al (1996) also reviewed the original eight PEMEX wells drilled in and around the Chicxulub site (Fig. 1). They reported that three wells (C-1, S-1, Y-6) drilled near the center of the gravity anomaly, and presumably in the center of the crater, intercepted crystalline silicate melt rocks (Fig. 3). It is interesting to note, however, that Sharpton et al. (1996) failed to include the 6–8 m of anhydrite and limestone at the base of the melt layer near the bottom of well Y-6 in their discussion and in their diagram (their Fig. 3).
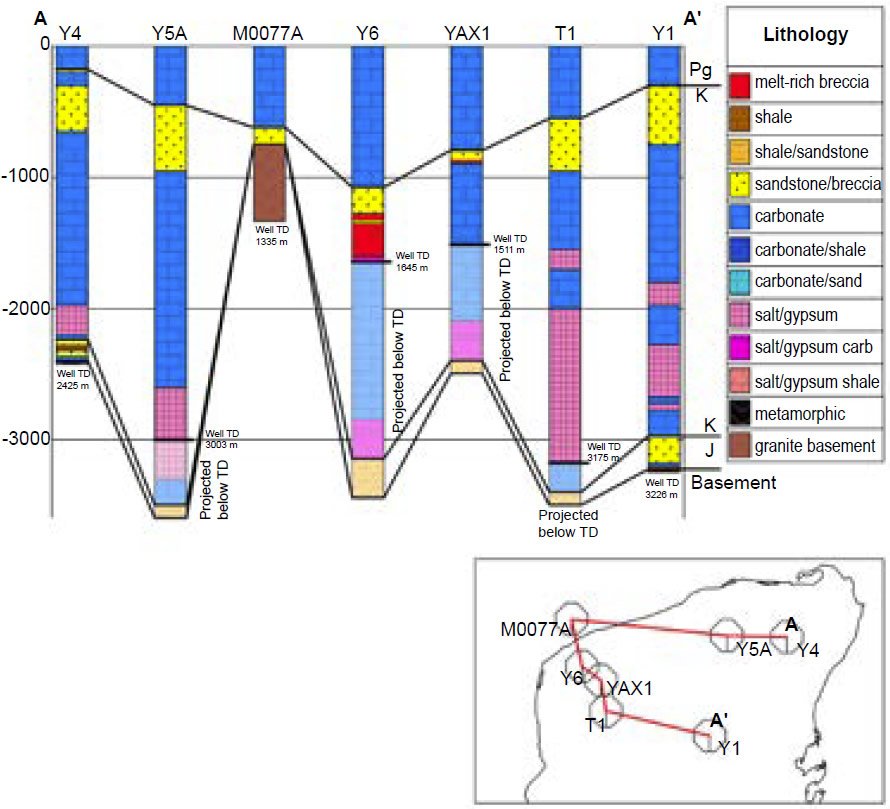
Fig. 3. Stratigraphic well and core data across the Chicxulub site. All depths are in meters. Locations of selected wells are also shown in Fig. 1. Wellbores Y5A, Y6, YAX1, and T1 have projected lithologic data below the TD point in each borehole. These projections are based on the surrounding wells and are colored a lighter hue. Note there is 6–8 m of sedimentary anhydrite just above the TD of well Y6, and below the melt-rich zone, implying a full sedimentary section may exist beneath as shown. Wellbores Y4 and Y1 reached metamorphic basement rocks and M0077A drilled over 587 m of granite. Not all boreholes were used in this diagram as C-1 was nearly identical to Y-6 and Y-2 was nearly identical to T-1. There was no published information available for well S-1. Figure prepared by Davis J. Werner.
Subsequent discoveries of suevite breccias, ejecta deposits and melt-matrix breccias, collectively termed impactites, in many well cores around the crater have led to claims that the impact origin for Chicxulub has been verified (Sharpton et al. 1996). Suevite is a rock deposit that contains deformed melt rock clasts and glass (Sharpton et al. 1996). In addition, features thought to be diagnostic of an impact were found in microscopic studies of the core rocks, including planar deformation features (PDFs) in quartz, feldspar and zircons, diaplectic quartz glass and fused mineral melts and whole-rock melts (Sharpton et al. 1996).
Christeson et al. (2001) conducted a geophysical study of the Chicxulub site using seismic lines shot in 1996 by the British Institutions Reflection Profiling Syndicate. They concluded there was a shallow (1 km thick) Tertiary (Paleogene) basin trending NE-SW, finding the edge coincident with a steep gravity gradient (Fig. 2). Furthermore, the southernmost edge of the shallow basin appears to coincide quite remarkably with the margins of the Chicxulub crater gravity anomaly along its southern end (Fig. 2). These authors also found that the Tertiary basin extended another 100 km to the north-northeast beyond the Chicxulub crater site (Fig. 2).
Christeson et al. (2001) also identified a low velocity layer of about 1 km thickness below the Tertiary basin over the center of the Chicxulub site which they believe to be a melt-rich layer. However, the three wells that penetrated melt-rich suevite layers (C-1, S-1, Y-6) only encountered 100–300 m of suevite (Fig. 3).
A large basement uplift was identified under the center of the Chicxulub site. Christeson et al. (2001) termed this feature the Central Uplift (Fig. 4). It was estimated to be 40–60 km wide with a vertical relief of at least 9 km. Christeson et al. (2001) also identified a larger basement uplift just to the northwest of the Chicxulub site (Figs. 2, 4) which coincided with, and likely is the cause for the large gravity high on the northwest flank of the presumed crater. This larger uplift, which was determined to be approximately 100 km wide, has a nearly identical amount of vertical relief (over 9 km) as the Central Uplift beneath the Chicxulub site (Fig. 4) (Christeson et al. 2001). Sharpton et al. (1996) suggested that Chicxulub gravity anomaly is not completely circular, as would be expected, because it is obscured by the larger gravity signal from this more massive basement uplift to the northwest (Figs. 2, 4).
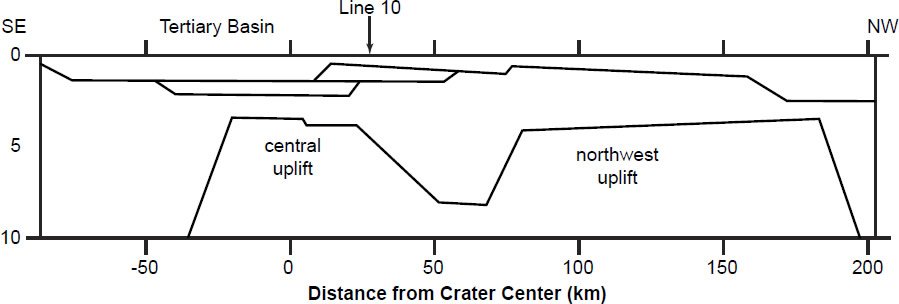
Fig. 4. Final density model of Christeson et al. (2001) showing the Central Uplift and a similar sized, northwest uplift along Chicx-B/F profile. Location of profile is shown on Fig. 2. Modified from Christeson et al. (2001). Figure courtesy of Susan Windsor.
Interestingly, the larger basement uplift to the northwest and the full extent of the shallow Tertiary basin to the northeast, and their respective corresponding gravity signals, are often not included in maps and diagrams of the Chicxulub site. Instead, many authors choose to cut off their maps of Chicxulub abruptly to the north, minimizing the effects of both features (i.e. Keller et al. 2004a). Some authors fail to show either of these features in their diagrams (i.e. Morgan et al. 2000).
From these seismic data and earlier well core studies, the presence of multiple circular slump blocks extending outward from the crater center (multi-rings), a collapsed central uplift and a peak ring that was presumably thrust over the top of sedimentary layers were interpreted (Morgan et al. 2000).
Additional seismic data were collected over the Chicxulub site in 2005 as part of the R/V Maurice Ewing cruise (Gulick et al. 2013). These new data, although rather poor quality below the K-Pg boundary (Fig. 5), have been interpreted to confirm the peak ring and the presence of melt and impactites over the Central Uplift.
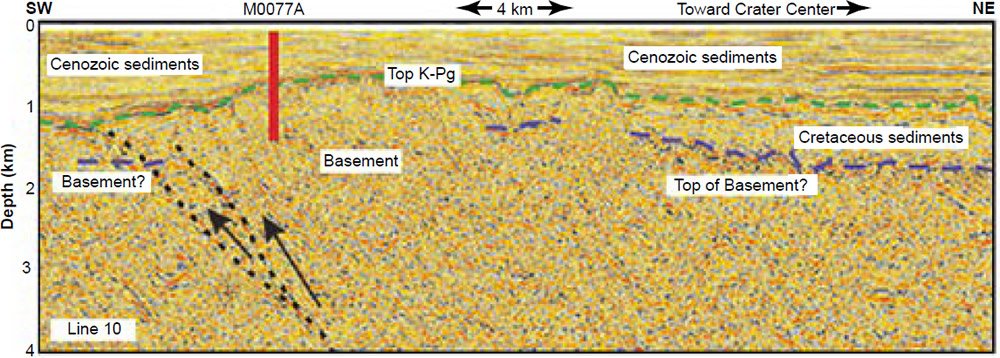
Fig. 5. Seismic Line 10 showing an alternative, basement thrusted interpretation as discussed in text. The location of borehole M0077A is also shown. Location for Line 10 is shown on Fig. 2. Seismic data is open access. Interpretation modified from Gulick et al. (2013). Figure courtesy of Susan Windsor.
In 2001–2002, and again in 2016, the International Continental Scientific Drilling Program (ICDP) drilled and collected cores from two sites at the Chicxulub location. The first well, called the Yaxcopoil-1 (Yax-1, Fig. 1), was drilled in the expectation of penetrating the impact melt lithologies and the underlying target rocks and to further detail the structure of the crater (Kring et al. 2004). A secondary goal was to refine the timing issues associated with the impact (Keller et al. 2004b).
The Yax-1 found about 100 m of combined melt-rich rocks and brecciated rocks (collectively referred to as impactite), about 1/5 the thickness of the nearby Y-6 well (Kring et al. 2004). It was further noted that the melt-rich layer is compositionally quite unlike melt-bearing impactites at other terrestrial craters (Kring et al. 2004). The melt-rich rock layer itself was only about 24 m thick and was found near the base of the overall 100 m-thick impactite zone. The brecciated layer contained clasts of dolomite, anhydrite, limestone, sandstone, and basement rocks like granodiorite, gneiss, and schist (Keller et al. 2004b). Devitrified glass spherules were also observed in the brecciated zone.
The 24 m-thick, green andesitic melt layer (López-Ramos 1975; Stinnesbeck et al. 2004) in the Yax-1 well was generally massive in appearance, aphanitic and dominated by microcrystalline pyroxene, plagioclase and alkali feldspar (Kring et al. 2004). The andesitic lithology was similar to the melt-rich layers encountered in earlier PEMEX wells (Y-6, C-1, S-1), and the pyroxene and plagioclase components of the melt were very similar to the composition of the melt rocks in the nearby Y-6 well (Kring et al. 2004).
The melt-rich layer in the Yax-1 well has also been heavily affected by hydrothermal activity, and the minerals having been altered at a temperature exceeding 300° C (Zürcher and Kring 2004). Because the melt layer was so thin in the Yax-1 well, Zürcher and Kring (2004) speculated that the thermal front for hydrothermal alteration must have come from somewhere else in the crater where the impact melt was thicker. However, as will be discussed below, there appears to be no thick impact melt anywhere within the Chicxulub site. Below the 100 m-thick impactite layer, the Yax-1 drilled into and bottomed in Cretaceous carbonate rocks. The hydrothermal alteration remains poorly explained by the impact theory and another cause, possibly from magmatic activity, is more likely.
The second well drilled by the ICDP (M0077A) was located offshore on the northwest flank of the Chicxulub site. (Fig 1). The well encountered carbonate-rich sediments (Paleogene) from depth of 505–618 m, then breccia and melt-rich rock from 618–748 m (Fig. 3). At 748–1335 m, the well encountered, and bottomed in, fractured granitic rocks with multiple pre-impact dikes and several impact-generated dikes (Morgan et al. 2016). Unlike the other wells at the Chicxulub site, M0077A did not drill through any Cretaceous carbonate rocks above the granitic basement (Fig. 3). The authors of the initial report on M0077A claim the encountered rocks confirm the dynamic collapse model (Morgan et al. 2016). They further claim that they drilled into the peak ring composed of basement rocks that were thrust upwards several kilometers and transported more than 20 km horizontally over sedimentary rocks, according to their dynamic collapse model (Morgan et al. 2016). However, as discussed below, there are alternative explanations to what was encountered in this well.
Specific Problems with the Chicxulub Site
This discussion will address some of the so-called diagnostic indicators of an impact, such as evidence of high pressure conditions and chemical indicators like iridium and high pressure minerals (French and Koeberl 2010). In spite of the claims by many secular scientists, there remain a number of scientific oddities about the Chicxulub site. Some of these involve the signatures of things that are more commonly found at other impact sites, but are not found at the Chicxulub site. Other oddities include non-diagnostic features that are perceived to be caused by an impact such as the peak ring, but also have alternative geological explanations.
Lack of an iridium anomaly
The secular model predicts a layer containing higher than crustal levels of iridium (a rare earth element) should spread out across the globe after a major impact hits the earth, but this is not necessarily the case. Much of the iridium produced in an impact stays in the debris at or near the impact site, and does not go up in a dust cloud to later settle globally (Taylor 2007).
Although higher levels of iridium have been found in rocks near the K-Pg boundary at many sites around the globe (Claeys, Kiessling, and Alvarez 2002), it is not universally distributed. In fact, Ir-anomalies are not always observed in adjacent locations within the same rock unit, as Clemens and Hartman (2014) found in the Hell Creek Formation in eastern Montana.
Sarjeant and Currie (2001) further reported that the original site in Gubbio, Italy, made famous by the Alvarez et al. paper (1980), was reanalyzed by Crocket et al. (1988) and Rocchia et al. (1990). These authors found that the reported iridium anomaly was not a single spike in value as claimed by Alvarez et al. (1980), but a series of iridium-rich layers spread across a 4 m thick interval. Sarjeant and Currie (2001) concluded that this is hardly the type of data that support a single impact.
And what was found at the Chicxulub site in all the cores and drilling results to date? Although a few traces of iridium were identified in melt rocks in wells C-1 and Y-5 (Fig. 1) (Schuraytz et al. 1996; Sharpton et al. 1992) no significant amounts of iridium have been found in any of the ejecta material or impactite layers across the Chicxulub site (Keller et al. 2004b). The amount found in the melt-rich rocks in C-1 and Y-6 ranged from none detected to 13.5 ppb, reportedly “well above typical crustal concentrations” (Sharpton et al. 1992, 820). Finally, there was no trace of an iridium spike in the top of the impactite in the Yaxcopoil-1 (Yax-1) core and no iridium reported to date within the M0077A core drilled in 2016.
In addition to the less than expected iridium, Paquay et al. (2008) found that the osmium residue in marine sediments at the K-Pg was insufficient for a large impactor the size of what has been claimed for Chicxulub.
Ironically, an iridium-rich layer is often used to identify the K-Pg boundary, where it is found (Claeys, Kiessling, and Alvarez 2002), and yet there is virtually no iridium in the impactite material at the very site claimed to be the “smoking gun.”
Shocked quartz not just from impacts
Shocked quartz is one of the more difficult discoveries at the Chicxulub site to try and explain using some endogenic terrestrial process other than an impact, but large volcanic eruptions and rapid magma quenching may provide possible answers. Shocked quartz is one of the high pressure induced transformations of the mineral quartz. It results in planar deformation features (PDFs) that are visible under an optical microscope (Huffman and Reimold 1996). Its presence in rocks is generally assumed to indicate an extraterrestrial impact occurred as quartz can only produce PDFs at pressures exceeding 10 GPa (100 kbars) (Gucsik 2009). Koeberl and Reimold (1999) have also suggested that identifying multiple directions of PDFs in a single quartz crystal is definitive proof of an impact, claiming that they can only form as a result of a hypervelocity strike.
However, this is apparently not true. PDFs and even multiple PDFs (up to 11 sets from seven quartz crystals) were found in samples from the Toba volcanic caldera, Indonesia (Alexopoulos, Grieve, and Robertson 1988).
Others have been critical of volcanically-derived PDFs, claiming the resultant PDFs are not as well formed or as planar as impact-derived PDFs, allowing the discrimination between volcanic and impact PDFs (Alexopoulos, Grieve, and Robertson 1988). But, Carter et al. (1986) found that the volcanically derived PDFs from Toba caldera had sharp, well-defined and continuous PDFs that more closely resembled the shocked quartz from a well-documented impact site (Mistastin crater). Lyons et al. (1993) reported that the spacing, shape, multiplicity, and orientation of PDFs do not reveal the nature or the source of the stresses responsible for their formation. All that is required for PDFs to form in quartz is the proper high pressure conditions.
Shocked quartz can even be created in the outer shell of fulgurites as the temperature and pressure of the groundmass is elevated in a lightning strike, resulting in shock metamorphic effects from pressures of about 25 GPa (Ende et al. 2012; Saikia, Parthasarathy, and Borah 2015). The presence of shocked quartz and PDFs is not sufficient to claim an impact has occurred.
Furthermore, Huffman and Reimold (1996) described several conditions where the development of over-pressured conditions in magmatic systems were sufficient to create PDFs, including quench supersaturation, catastrophic phase changes and gas-phase explosions. Quench supersaturation involves a very specific type of closed magma system where dissolved volatiles are able to reach supersaturation prior to eruption. In this scenario, a pressure front is generated across the magma chamber once crystal nucleation begins, triggering further exsolution of volatiles and crystallization as the magnitude of the pressure front increases (Huffman and Reimold 1996). Rice (1985, 1987) suggested peak pressures above 50 GPa are possible in this situation.
A second way to generate a pressure front is through catastrophic phase transitions (Huffman and Reimold 1996). Experimental petrology has demonstrated the ability of natural silicate systems to produce large amounts of gas rapidly enough to initiate a shock wave across the magmatic chamber as the melt solidifies (Boslough 1989; Rabie, Fowles, and Fickett 1979). However, Huffman and Reimold (1996, 210) warn that “such detonations involving rapid crystallization will require rapid chemical transport or shock-induced shear transport.”
A third method of generating a pressure wave is through gas-phase explosions. Huffman and Reimold (1996) describe conditions where dissolved gasses can change dramatically during rapid ascent and volcanic eruption. Under the right conditions and the presence of explosive gases, catastrophic gas reactions can contribute to a propagating, shock-induced wave front passing through the magma (Huffman and Reimold 1996).
Most of the shock-generating conditions, described by Huffman and Reimold (1996), involve rapid ascent and rapid crystallization of magmas which they believe to be uncommon. However, the rapid ascent of magmas seems to be much more common than petrologists once believed. Rapid vertical ascent of magma has been noted in several newer, secular studies. Terry Plank and Philipp Ruprecht determined that the magma beneath Costa Rica’s Irazú volcano rose roughly 35 km in just months, or at most a few years (Rosen 2016), finding that some magmas can ascend 10 km in just ten minutes.
Other studies have found that magma beneath ocean ridges can rise over 5 km at rates exceeding 1.0 m/sec (Tan et al. 2016). Plank and Ruprecht further suspect that rapidly moving magmas are related to the explosivity of the volcanic eruption because they release their dissolved gases more quickly compared to slower ascending magmas (Rosen 2016). These recent studies demonstrate that rapid phase changes due to rapidly ascending magmas may well be the norm. Shock waves generated by these reactions may be the cause of some of the shocked quartz we observe, even within intrusive igneous rocks.
The uniform shock pressures found throughout the over 500 m of felsic basement rock in well M0077A (Fig. 3) also are better explained by a magmatic origin such as a catastrophic phase change. Morgan et al. (2016) found no systematic change in shock metamorphism with depth of penetration in the basement rocks. All minerals showed shock pressures of about 10–35 GPa.
Limited high pressure mineral forms
Morgan et al. (2016) suggest that the so-called peak ring rocks were subjected to shock pressures of 10–35 GPa, while the melt rocks were subjected to pressures in excess of 60 GPa. Oddly, they found no indications of a systematic variation in depth within the coarse-grained, granitic basement “target rock” in drill hole M0077A, where over 500 m of basement was penetrated and cored. These findings suggest a uniform high pressure penetrated through the felsic rocks, fitting better with a pressure wave interpretation caused by rapid phase changes and crystallization as described above.
Pressures approaching or exceeding 60 GPa are also capable of forming high pressure minerals, not just PDFs, that can be indicative of an impact as they have been found at other known impact sites. Gucsik (2009) lists coesite (pressure range of 30–60 GPa) and stishovite (pressure range of 12–45 GPa), two high-pressure polymorphs of quartz that form under shock metamorphism. Coesite has been reported in 15 terrestrial impact craters in crystalline and/or mixed sedimentary and crystalline target rocks, and stishovite in about half as many craters (Grieve, Langenhorst, and Stöffler 1996). The common presence of these high pressure mineral forms would go a long way in documenting diagnostic high pressure conditions indicative of an impact (French and Koeberl 2010).
Gucsik (2009) also lists diaplectic quartz glass, a solid-state transformation product of quartz formed at pressures above 35 GPa, and lechatelierite a liquid-state transformation product of quartz formed at pressures above 50 GPa, as additional minerals potentially indicative of a meteorite impact. However, lechatelierite can only occur under conditions of whole-rock melting (Grieve, Langenhorst, and Stöffler 1996). Diaplectic glass and lechatelierite are less commonly identified in rocks associated with an impact compared to PDFs, but are still good indicators of high pressure conditions (Grieve, Langenhorst, and Stöffler 1996).
And yet, few of these high pressure minerals have so far been identified in the studies of the drill cores at or near the Chicxulub site. Kring et al. (2004) report finding a silica clast with ballen structure indicative of cristobalite in the Yaxcopoil-1 (Yax-1) well. Ballen quartz alone cannot be used as direct evidence of shock metamorphism, only indirect evidence (Ferrière and Osinski 2013). And cristobalite is a low pressure, high temperature quartz polymorph that is present in many siliceous volcanic rocks and is not usually indicative of shock metamorphic conditions (Hurlbut and Klein 1977). Tuchscherer et al. (2004) reported finding ballen quartz within the Yax-1 well core. They suggested it could be the result of recrystallization of diaplectic glass, indicating a possible shock pressure of about 30 GPa (Tuchscherer et al. 2004). However, they did not actually identify diaplectic glass, or at least it was not mentioned in their paper. Sharpton et al. (1996) did find some diaplectic glass in the impactite layers of PEMEX wells Y-6 and S-1 (Fig. 1) but did not quantify the amount.
Stishovite and lechatelierite have also not been conclusively identified in the any of the studies of the Chicxulub well cores. However, Sharpton et al. (1996) suggest that some of the ballen structures they found may have come from devitrification of lechatelierite. Admittedly, these minerals are more difficult to identify optically, with stishovite appearing as concentrations between PDF lamellae (Grieve, Langenhorst, and Stöffler 1996).
Only two fragments from one well core (Y-6, Fig. 1) at the Chicxulub site yielded coesite-bearing quartz grains (Lounejeva, Ostroumov, and Sánchez-Rubio 2002). The presence of coesite and PDFs in the same quartz grain indicates a pressure between 30–35 GPa was likely achieved for these rocks (Grieve, Langenhorst, and Stöffler 1996; Lounejeva, Ostroumov, and Sánchez-Rubio 2002). Stöffler (1971) has stated that rocks from large craters should be expected to exhibit higher contents of coesite and stishovite than those of smaller craters. This makes their limited identification at Chicxulub all the more puzzling.
Few shatter cones found
Shatter cones can also be used as an indicator of an impact and have been found in about half the confirmed impact structures, where they are usually in rocks from the central crater and sometimes as fragments in the impact breccias (Ferrière and Osinski 2013). Shatter cones are thought to form at fairly low pressures (2–10 GPa) and possibly early in the impact process. However, Ferrière and Osinski (2013, 108) also readily admit that, “The formation of shatter cones, widely accepted as unequivocal proof of a meteorite impact crater, is still not completely resolved.” They further acknowledge that shatter cones can be difficult to distinguish from non-impact features like cone-in-cone structures and slickensides.
Considering all the detailed studies conducted on the cores at Chicxulub, it seems rather odd that the only mention of shatter cones is in the most recent paper, from the last well drilled (M0077A). Morgan et al. (2016) observed a few shatter cone fragments in pre-impact dikes within the granitic basement rocks and also within the impact breccia. The impact breccia, target rocks, and melt rocks at the Yax-1 borehole failed to turn up any shatter cone structures even after intense scrutiny by numerous working groups (Keller at al. 2004a, 2004b; Kring et al. 2004; Tuchscherer et al. 2004; Zürcher and Kring 2004). And there is no mention of shatter cones in any of the studies of the earlier drilled wells by PEMEX (Sharpton et al. 1996; Ward et al. 1995).
Very little pseudotachylyte
Pseudotachylyte (PST) is a special type of cataclasite rock that contains some frictional melt and is caused by catastrophic faulting (Spray 1995). Faulting caused by ring collapse of impacts, like Chicxulub, are expected to generate large volumes of melt in the ring fault zones, resulting in extensive production of PST (Morgan et al. 1997). Rings of PST have been identified at the Sudbury crater, Ontario and interpreted as a consequence of catastrophic faulting during the formation of a large, multi-ring crater (Morgan et al. 1997). Because PST can also be produced by other types of tectonically-driven, catastrophic faulting, it cannot be used as sole confirmation of an impact (Spray 1995).
Several authors reportedly identified PST along impactite dikes and melt rocks in the lower part of the Yax-1 core (Kenkmann et al. 2003; Smit et al. 2002; Wittmann et al. 2003). Smit et al. (2002) reported “numerous pseudotachylyte veins and dikes of suevite and of melt breccia” in the Cretaceous sedimentary rocks near the base of the Yax-1 well. They believe the PST is related to ring faulting caused by the Chicxulub impact. Unfortunately, there are no published images of the claimed PST as all three references above refer to abstracts from society meetings.
However, no other reports of PST were noted by other authors who studied the Chicxulub boreholes. The limited amounts of PST seem confined to the Yax-1 borehole and only to the pre-impact rocks (Cretaceous). A reexamination of the Yax-1 borehole by Stinnesbeck et al. (2004) found only two injection veins and just two dikes of suevite (containing some melt remnants) in the borehole. These authors did not identify PST within the veins or the dikes. Instead, they interpreted the injection veins as a “black fine-grained bituminous dolomite with intraclasts of anhydrite, calcite, pyrite and clay minerals” (Stinnesbeck et al. 2004, 1054). They believe the injection veins were formed by the forcing of sediment and hydrothermal fluids into cracks and/or shear zones in the Cretaceous dolomite.
The small amount of melt
Most studies of the so-called melt rock in the Y-6 well (Fig. 1) concluded it is a green, fine-grained andesite filled with glass, melt-rock clasts, and fragments of rocks such as granitic gneiss (Hildebrand et al. 1991; Kring and Boynton 1992; Sharpton et al. 1992, 1996). Kring and Boynton (1992) further argued that the melt observed in the Y-6 well could not have formed from normal volcanic differentiation as “the liquid represented by the augite-bearing groundmass does not lie on chemical trends produced by igneous fractionation processes.” However, most of their comparison rocks were from subduction zone magmas and not volcanic rocks that may have mixed with other non-magma sources, including the basement rocks and sedimentary cover rocks found in the Yucatán Peninsula (Tuchscherer et al. 2004).
Further, Kring and Boynton’s (1992) argument that only an impact could produce the observed melt mixture containing basement and sedimentary components is unfounded. An igneous intrusion originating in the basement would likely melt and assimilate some of the country rock as it migrated, changing the composition of the melt from a purely magmatic source as discussed above. In fact, the melt-rich rocks at Chicxulub were found to contain inclusions of granite, schist, gneiss, amphibolite, carbonate minerals, and anhydrite (Tuchscherer et al. 2004). And Sharpton et al. (1996, 64) concluded that the “melts were derived exclusively from continental crust and platform sediment target lithologies, with no evidence of a significant mantle or oceanic crustal signature.” It is no wonder these melt rocks fail to follow normal volcanic melt fractionation curves. The atypical, assimilated composition of the magmas should not be expected to mimic normal igneous behavior.
Early estimates of melt volume were based on calculations of a transient crater of 110 km in diameter (Hildebrand et al. 1991). Kring and Boynton (1992) calculated a melt volume of between 3.7 × 104 km3 and 1.0 × 105 km3 of total melt if scaled using other impact craters. Melt volumes were later reduced slightly to 1.0−2.0 × 104 kmr (Pope, Kieffer, and Ames 2004). Nonetheless, Morgan et al. (2000) anticipated thick melt beneath the central portion of the Chicxulub crater (their Fig. 3) as much as 3–4 km thick based on geophysical data (Kring et al. 2004). And the dynamic collapse model of Morgan et al. (2016) predicts the creation of tremendous amounts of melt in the central crater region, with over 1 km spread across large portions of the crater (their Fig. 1). Gulick et al. (2013) found that the seismic data across the site could not properly image nor constrain a melt sheet, leaving the estimated amount of melt very subjective.
The wells drilled into the Chicxulub structure tell us a different story, however. The thickest melt encountered in any of the wells was between 100–300 m, and several penetrated far less or not at all (Christeson et al. 2001; Kring et al. 2004; Morgan et al. 2016). Kring et al. (2004, 894) lament that “The total amount of impact melt is, thus, unusually small in this core [Yax-1] for a crater the size of Chicxulub, some 180 km in diameter.” In order to explain the lack of melt in Yax-1, Kring et al. (2004) suggest that the well was drilled on some sort of topographic high which prevented the deposition of thicker melt. However, the wells drilled into the Chicxulub structure have not found any significant melt as anticipated (Fig. 3).
In the central portion of the Chicxulub structure, wells C-1 and S-1 bottomed in thin melt layers of less than 300 m that conceivably could be thicker (Sharpton et al. 1996). However, the nearby well Y-6 puts a limit on the melt thickness in the center of the structure as it encountered 6–8 m of laminated, anhydrite-rich sedimentary rock at the bottom of the well, below the melt-rich layer (Hildebrand et al. 1991) (Fig 3), tightly constraining the thickness of the melt near the center of the presumed crater.
The well data are compelling. There is not nearly enough melt encountered in the wells at Chicxulub to fit the amount of melt expected or modeled by secular scientists for such a presumed massive impact. These results indicate that something is wrong with the models.
Unfounded claims of a peak ring
The most recent contribution in the Chicxulub saga reported on the drilling results of the M0077A well site (Morgan et al. 2016). In their paper, the authors claim to have confirmed the presence of the peak ring after drilling into crustal rocks along the edge of the crater site. And yet, they failed to drill through the crustal rocks and into deeper sedimentary layers in order to truly confirm their hypothesis. In fact, the seismic line (Chicx-R3, Fig. 5) they show through the drill site is rather poor in quality and can be interpreted in multiple ways (Morgan et al. 2016, Fig. 1G). The reflectors shown are nearly all discontinuous and it is only their subjective shading of the data that reveals the interpretation they desire. It is just as likely there are few, if any, sedimentary rocks beneath the drill location, and more likely just more granitic crustal rocks instead.
Contrary to their second claim, Morgan et al. (2016) also failed to confirm their dynamic collapse model as illustrated in their Figs. 1A-F. This model predicts copious amounts of melt generated from the impact and significant, dynamic deformation of the crustal rocks down to depths of 30 km. They further claim that the granite they drilled into in core M0077A was part of the mid-crustal basement that was transported upward by the impact at least 3 km and possibly 8–10 km (Morgan et al. 2016). Thus, they argue, they confirmed their model by tagging granite beneath the breccia layer. But, just because they found granite crust beneath the breccia layer underneath the Paleogene sediments does not prove they drilled into the so-called peak ring.
Their dynamic collapse model predicts tremendous deformation of the basement rocks occurring in the first ten minutes following the impact (Morgan et al. 2016, Figs. 1A-F). This amount of deformation would most likely have resulted in ductile flow throughout the basement rocks, probably producing a pervasive rock fabric and the development of a dominant direction of foliation. Surely there should have been evidence of some type of internal deformation of the minerals (evidence of plastic flow) or evidence of ductile flow or at least some fabric trajectories produced by the movement of tens of kilometers of felsic basement rock (Ramsay and Huber 1983). And yet, the authors illustrate and describe what appears to be exclusively brittle deformation effects and simple fractured felsic basement rock (Morgan et al. 2016, Fig. 2). They even identified pre-impact mafic and felsic dikes in the core that are undeformed and unfolded, merely fractured (Morgan et al. 2016). Surely these structural features should have been highly deformed during the presumed several kilometers of transport upward and over the side of the crater, creating the so-called peak ring. It is a wonder, in their suggested scenario, that any pre-impact dikes are identifiable at all.
Instead, what the core at M0077A drilled was likely a faulted basement uplift that brought felsic crystalline rocks closer to the surface. From the gravity and the seismic data, it appears that the M0077A well drilled into the southwestern flank of the larger basement uplift northwest of the Chicxulub site (Figs. 2, 4, 5). This structure is the likely origin of the gravity high that is so often ignored in any discussion of the claimed impact.
Admittedly, the other two wells that reached basement rocks near the Chicxulub site, PEMEX Y-1 and Y-4, reached quartz chlorite schist and extrusive rhyolite below the lowest sedimentary units and not granite (Fig. 3) (Kring et al. 2004). However, in the southern part of the Yucatán peninsula, another well did reach granite (Kring et al. 2004). This well also reportedly had Permian-Triassic granitic intrusions that penetrated the basement and the overlying sediments (Kring et al. 2004). Additionally, the lack of any metamorphic rocks on top of the deeper granitic basement rocks in M0077A may only be due to erosion across the uplift prior to the deposition of later sedimentary rocks.
Therefore, the discovery of granite in the lower part of core M0077A is not confirmation of the dynamic collapse model that claims to have brought granite upwards several kilometers (Morgan et al. 2016). The well did not drill into sedimentary rocks underneath the granite. It merely drilled into the variable basement rocks of the Yucatán Peninsula. And most likely drilled into a basement uplift as indicated by the gravity data (Fig. 2).
Large tsunamis not likely from the Chicxulub impact
Many authors have proposed that the presumed Chicxulub impact would have generated devastating tsunamis across the Gulf of Mexico, the Caribbean, and the Atlantic basins that were up to 100–300 m high and penetrating up to 300 km inland (Kring 2007). This hypothetical scenario prompted the search for tsunami-generated layers across the Caribbean and Gulf of Mexico region. And sure enough, coarse deposits, claimed to result from tsunami waves caused by the Chicxulub impact have been identified all across the Gulf of Mexico region at or very near the K-Pg boundary (Bourgeois et al. 1988; Gale 2006; Kring and Boynton 1992; Schulte et al. 2006; Smit et al. 1996; Smit 1999). Because many of these conglomeritic rocks, referred to as polymictic breccias, contained similar constituents, like higher levels of iridium, and impact spherules and melt fragments, they were quickly assigned to the Chicxulub impact. These same breccias are sometimes classified as suevite breccias due to the presence of melt-rich clasts (Sharpton et al. 1996).
Several authors have also pointed out that these suevite breccias and/or conglomerate beds seem to thicken toward the Chicxulub site, using data from Cuba, Haiti, Mexico and the Gulf Coast of the United States (Alegret et al. 2005; Grajales-Nishimura et al. 2000; Kring 2007). The origin of this so-called “clastic megabed” (Alegret et al. 2005) has been linked to the Chicxulub impact as it resides at or near the K-Pg boundary. However, the limited number of sampling locations across this vast of a region makes exact correlations problematic. In addition, Koeberl (1993) studied the geochemical signatures of the Haiti impact glasses, comparing them to Chicxulub target rocks (and even the breccia layer in the Y-2 well), and found them to be compositionally different. Koeberl concluded “that there are still questions regarding Chicxulub as the source for the Haitian K-T [K-Pg] boundary glasses” (Koeberl 1993, 214).
And so-called polymictic breccias are not unique to impacts, as popularly believed. They are merely defined as clastic rocks with many types of minerals or rock types. In fact, a polymictic breccia has been identified within the Middle Jurassic La Joya Formation in central Mexico which contained volcanic, sedimentary, and metamorphic clasts, including white, hydrothermally-altered quartz (Barboza-Gudino, Molina-Garza, and Lawton 2012). These polymictic breccias are interpreted by secular geologists as alluvial fan deposits, and are not associated with any impact.
Finally, Boslough et al. (2016) recently modeled the effects of an asteroid impact in water. They found through a 3D computer simulation that an impact would likely not cause a devastating tsunami across a broad region. They concluded that an asteroid impact acts as a point source and only affects the immediate vicinity surrounding the impact location. They correctly pointed out that a true, large-scale tsunami is only generated when something impacts the entire water column, like vertical movement of the seafloor.
It is possible then, that the so-called tsunami deposits identified by so many secular geologists are not tsunami deposits at all, at least not generated by a single asteroid impact. Unfortunately, an impact origin for these coarse-grained deposits is so ingrained in the thought patterns of these secular authors that they cannot fathom any other possible cause for these clastic deposits. They insist, in spite of the findings of Boslough et al. (2016), that there must have been a large impact and huge tsunamis to generate these deposits across the vast region of the Gulf and Caribbean Sea.
Questioning the timing of the impact
Although Gerta Keller and her colleagues do not question an impact origin for the Chicxulub structure, they have for many years questioned the timing of the presumed impact, claiming it cannot be the cause of the dinosaur extinction as the timing is off by as much as 300,000 years (Keller and Adatte 2007; Keller et al. 2004a, 2004b, 2007; Stinnesbeck et al. 2004; Ward et al. 1995).
The main discrepancy in the timing debate involves a 50 cm thick, laminated micritic, and partially dolomitized limestone above the suevite layer (impact breccia and/or melt) in the Yax-1 well. Keller and her colleagues interpret this section as uppermost Cretaceous age based on paleontologic, isotopic, mineralogic, and magnetostratigraphic data, whereas most other authors believe it to be a reworked upper impactite layer that is nearly coincident in time with the impact event (Kring 2007).
Keller and her colleagues make a fairly compelling argument based on the geologic data. Stratigraphic boundaries should be consistently picked on observable changes in the rocks and changes in the fossils within the rocks. Following this line of reasoning, the presumed Chicxulub event does seem to have occurred prior to the end of the Cretaceous.
However, 300,000 years is much less of a problem than the recent shift in the secular age for the K-Pg boundary which moved the date back by nearly one million years. Up until 2008, the “exact” age of the K-Pg boundary was firmly established at nearly exactly 65 Ma as was the Chicxulub event (Krogh et al. 1993; Sharpton et al. 1992).
Then, orbital tuning struck the age of the boundary like an asteroid. Kuiper et al. (2008) suggested a new standard for 40Ar/39Ar dating which changed the K-Pg boundary from 65 Ma to 66 Ma. This new standard was presumably calibrated to tephra layers within astronomically tuned open marine sediments in Morocco. Therefore, they claimed, all earlier “factual” ages of 65 Ma years were deemed wrong, regardless of methodology. And correspondingly, the age of the Chicxulub impact was likewise changed to reflect this time shift.
Hebert (2014, 2016a, 2016b, 2016c) has shown quite convincingly the circular reasoning involved in orbital tuning. In a series of papers, he pointed out the unaccounted for 80,000 year time shift in the methodology since the original publication of the “pacemaker paper” (Hayes, Imbrie, and Shackleton 1976), invalidating the accepted Milankovitch explanation, and the very basis of orbital tuning. And yet, secular science ignores these “minor” changes and continues to tell tales of deep time, asserting that the newer ages are better, more accurate and synchronized (Kuiper et al. 2008).
Discussion and Alternative Explanations
The original interpretations for the melt rocks at Chicxulub varied from Cretaceous intrusive igneous features, like a laccolith (Peterson 1983), to volcanic activity (extrusive andesite) (Guzmán and Mina 1953; López-Ramos 1975). Since 1991 however, the concept of a dinosaur-killing asteroid impact has become mainstream, based largely on a semi-circular gravity anomaly and to a lesser degree on magnetic data (Hildebrand et al. 1991). The formerly igneous and/or volcanic rocks penetrated by several wells have now become accepted as impact melts.
Although a gravity anomaly has been used to justify the impact, gravity data are extremely ambiguous and can have multiple solutions. Nearly unlimited combinations of densities can be combined to achieve the same net result (Telford, Geldart, and Sheriff 1990). Nonetheless, the circular nature of the gravity anomaly at Chicxulub has been used to model a large central impact crater about 170 km diameter with multi-ring faults extending out nearly 300 km from the center (Sharpton et al. 1993).
Most of the papers discussing the Chicxulub site only discuss the gravity data at the so-called crater site. The larger gravity high to the northwest is generally not discussed. It seems incredulous that the more significant gravity high (Figs. 2, 4) immediately to the northwest of the crater site has been systematically ignored, other than to use it as a reason for the incomplete, circular nature of the Chicxulub anomaly (Sharpton et al. 1993). The high gravity signal observed over this northwest feature implies that a basement uplift of some sort exists under this area, trending northwest-southeast (Fig. 4). And yet, the smaller gravity high at the center of the Chicxulub site, while on trend with the larger anomaly to the northwest, is claimed to be produced solely from an impact. If an impact is responsible for the smaller gravity high at Chicxulub, what mechanism produced the larger anomaly? A second impact? It seems far more likely that both were products of the same geologic activity as both are on trend with one another. Since the larger anomaly is not circular-shaped, it is less likely it was produced by an impact. Similarly, the central Chicxulub anomaly could just as easily be the result of basement uplift like the anomaly to the northwest (fig. 4).
Geologic proof often comes through the drilling of wells. And the last well drilled at the Chicxulub site, M0077A, bottomed out in over 500 m of felsic basement. It’s likely this well drilled into the flank of a faulted basement high, verifying, not a peak ring as claimed, but the presence of a basement-cored (granite) uplift northwest of Chicxulub. Seismic data collected across this basement trend show a reverse fault on the west side in support of a block-faulted interpretation (fig. 5).
Near point source gravity highs (positive above residual) are often surrounded by a correspondingly circular low, called a halo (negative below residual), similar to other types of wave data (Telford, Geldart, and Sheriff 1990). Therefore, a small, high density basement uplift (gravity high), roughly circular in nature, would produce a surrounding, roughly circular low trough (gravity low). The Chicxulub gravity anomaly could then be explained by the coincidence of two structural features, one shallow and one deep, and by the wave-like nature of gravity data, producing both the observable highs and lows. It is possible that the overlap of the southern part of the shallow Tertiary (Paleogene) basin identified by Christeson et al. (2001) with the southern end of the deeper, northwest-southeast-trending basement uplift system could produce the observed semicircular-shaped anomaly (fig. 2). In this scenario, the central basement uplift provides the deeper high gravity signal, while the surrounding low gravity halo and the shallower, Tertiary basin create the semi-circular low. The overlapping nature of shallow and deep features, similar to coincident heel prints, are simply added to produce the anomaly. The deeper structural trend was further confirmed by the M0077A well where over 500 m of granite was found at the bottom of the borehole. This well did not drill into the “peak ring” as claimed, but merely elevated basement rock (figs. 4 and 5).
And it is equally incredulous that the shallow Tertiary basin which extends to the north, beyond the Chicxulub site has also been largely ignored. Christeson et al. (2001) identified a shallow low-velocity sedimentary basin, filled with Tertiary (Paleogene) sediment, that trends northeast-southwest across the Chicxulub site and extends about 100 km northwest beyond the presumed impact location. They also noted that the steep edge of the basin correlates with the steep gravity gradient in some areas near the Chicxulub site (Christeson et al. 2001). Basin bounding faults and the claimed ring faults are identical in their nature and difficult to differentiate as both are normal faults with the hanging wall down. Either type of structure could be interpreted to create the basin and/or the crater. It is possible that the claimed ring faults that extend out 150 km (300 km diameter) from the center of the “crater” (Morgan et al. 1997) are just normal faults associated with this shallow basin. Unfortunately, most studies merely claim these are ring faults because they only concentrate on the southern part of the Tertiary basin, the section that overlaps the presumed impact crater. Because this shallow faulted basin extends well beyond the crater site, it is likely that basin-bounding faults continue northward also and that the “ring faults” are just a part of this Tertiary basin development. The gravity “low” associated with the outer part of the crater and the gravity “low” for the Tertiary basin to the north are nearly identical (fig. 2). The crater cannot be examined without the basin and vice versa.
In addition, the semi-circular pattern of cenotes (collapsed caves) and sinkholes identified by Connors et al. (1996) coincides quite nicely with the outer edge of the shallow Tertiary basin across the Yucatán Peninsula, as previously identified by Morgan et al. (1997). Basin-bounding normal faults that define the outer edge of the shallow structure would naturally serve as conduits for increased ground water flow. Indeed, it should be expected that fluid flow along shallow faults would cause more dissolution of carbonate rock and create a pattern of sinkholes and cenotes that reflect the fault trace. Therefore, the pattern of sinkholes and cenotes are not necessarily evidence of an impact. They only reflect a pattern of concentrated and increased fluid flow.
Finally, it is interesting to note that the rocks penetrated by the Yax-1 well were discovered to have experienced extensive hydrothermal alteration. Zürcher and Kring (2004) have proposed a massive melt source must exist to explain the degree of hydrothermal alteration observed. But where is the melt source they postulated? Melt is considered proportional to the size and velocity of the impact (Melosh 1989). Chicxulub should have produced lots of melt (Morgan et al. 2016). However, the well data tell a different story. The wells drilled into the Chicxulub structure only found limited amounts of melt-rich rock, or none at all, leaving the postulated massive melt-rich zone still missing. Hydrothermal alteration is not commonly associated nor reported with impact melts as they are likely produced too rapidly. It seems more likely that the hydrothermal alteration in the Yax-1 well was the result of intrusive igneous activity and not from an undiscovered massive impact melt. In contrast, superheated ground waters are commonly produced above and ahead of an intrusive magma body.
What about the large clastic megabed that has been found to contain glass, tektites and shocked quartz and is spread across the Gulf of Mexico region? The global Flood offers an alternative explanation. Vast deposits, sometimes called blanket sands, are fairly common in the geologic record and can be explained by the activity of the great Flood (Snelling 2009). And in particular, a basal Paleogene (Lowermost Tejas sequence) sandstone complex covers much of the Gulf of Mexico (fig. 6). Part of this basal sand includes the deep water “whopper sand” discussed by Clarey (2015) and is commonly referred to as the Lower Wilcox sand. Based on the extent of this blanket sand deposit, it should be no surprise that there are common components to the deposits at or near the K-Pg boundary. The great Flood would naturally spread the same minerals within the same sand layers across large regions as documented by Snelling (2009). There was sufficient Late Cretaceous and Paleogene volcanic activity in the subduction zone along western Mexico, and across Cuba and much of the Caribbean (Jamaica, Hispaniola, Puerto Rico, and the Dutch West Indies) to easily provide a source for the glassy spherules so commonly claimed to be exclusively from the Chicxulub event (Martini et al. 2009; Pardo 2009).
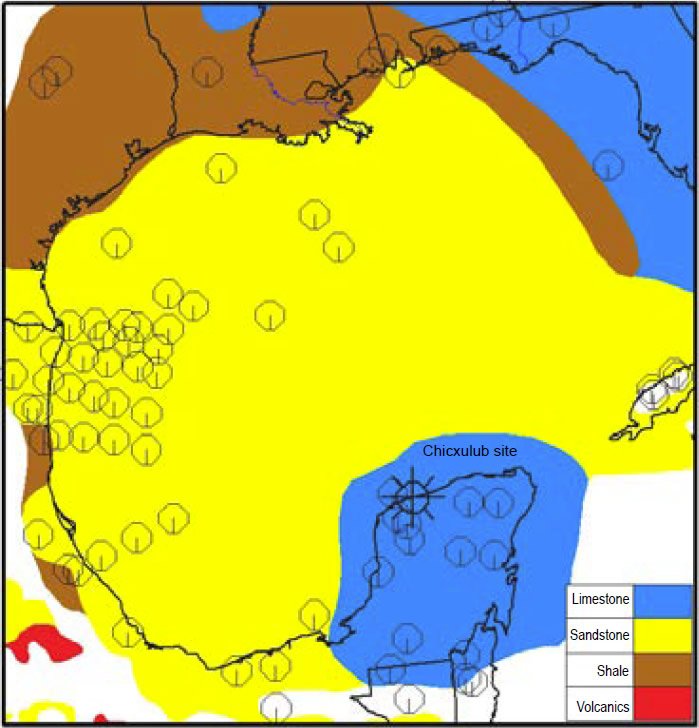
Fig. 6. Basal (lowermost) Tejas sequence lithology map of the Gulf of Mexico region, showing the extent of the “blanket sand” across most of the region (colored yellow). Circles shown represent locations of stratigraphic columns derived from borehole data, selected seismic data and/or outcrops. The basal Tejas across the Yucatán peninsula is colored blue (carbonate-rich) assuming the breccia and melt-rich rocks at Chicxulub are latest Cretaceous deposits (upper Zuni sequence), which may not necessarily be the case. These deposits could just as easily be correlated with the basal Tejas sand across the Gulf of Mexico, which includes the Whopper Sand (Clarey 2015). Figure prepared by Davis J. Werner.
What is the Chicxulub feature? If it was an impact, it seems to have been much smaller and less significant than generally assumed (Paquay et al. 2008). Although the data are not conclusive, a case can also be made that there was no impact at the Chicxulub site. Everything can be reasonably explained by volcanic/intrusive activity and the overlap of shallow and deep structural features. In addition, the intrusive body itself may be circular in shape, like a laccolith, further enhancing the circular nature of the gravity signal.
Conclusions
The Chicxulub impact has become the iconic tale that is readily accepted by most of secular science to explain a so-called major extinction event that included the dinosaurs. They need a story to explain these events in earth’s past primarily because they have no other recourse. Secular science categorically rejects earth history as described in the Bible, including the reality of the global Flood. In order to explain the disappearance of the dinosaurs and other creatures, they uphold the Chicxulub impact as one of the principle factors in their tale. Yet, the evidence for the impact at the Chicxulub site is not as compelling as claimed or generally assumed. Consensus science cannot justify the impact.
Some of the diagnostic criteria for identifying an impact have been found at the Chicxulub site, including the identification of shock-metamorphism and chemical signatures like iridium, shatter cones, PDFs, and high pressure minerals and glasses (French and Koeberl 2010). But, even these high pressure shock-metamorphism conditions and altered minerals can be caused by other circumstances, including rapid crystallization and volcanic activity. The questions about the timing of the impact, the thinner than expected melt-rich layer, the lack of any substantial iridium, the less than expected high pressure minerals, the lack of PST present in the cores, the relatively undisturbed Cretaceous sediments beneath the melt-rich lenses and the ambiguous nature of the gravity anomaly all raise concerns about the Chicxulub Crater, and ultimately, the asteroid extinction theory. The “burden of proof” has still not been met.
An impact may have occurred at the Chicxulub site during the Flood, but if so, it seems to have been smaller than commonly envisioned, creating merely a fraction of the postulated effects. And it is entirely possible there was never an impact at Chicxulub. And the latest research indicates that even the tsunami evidence claimed to exist around the Gulf of Mexico seems unlikely to be from the impact. All of the data have alternative explanations, including the shocked quartz, melt rocks, hydrothermal activity, and the structural and geophysical data. Unfortunately, without the discovery of meteorites, or traces thereof (French and Koeberl 2010), the data will likely remain inconclusive.
Several creation scientists have called on a shower of possibly 1000s of asteroids to have hit the earth during Creation Week (Spencer 2015) or at the onset of the Flood event (Oard 2014) or both. But the physical and geologic evidence for most of these impacts is lacking. As scientists, we must be careful to not invoke mechanisms without the support of sufficient data. Admittedly, some impacts likely occurred as part of the one-year global Flood. However, creation scientists do not think these caused any true extinctions as God protected Noah and the animals on the Ark, even from extraterrestrial impacts. But, because secularists ignore the evidence of a global Flood, they will continue to create stories and debate the causes of the K-Pg extinction for many years to come.
References
Alegret, L., I. Arenillas, J. A. Arz, C. Diaz, J. M. Grajales-Nishimura, A. Meléndez, E. Molina, R. Rojas, and A. R. Soria. 2005. “Cretaceous-Paleogene Boundary Deposits at Loma Capiro, Central Cuba: Evidence for the Chicxulub Impact.” Geology 33 (9): 721–724.
Alexopoulos, J. S., R. A. F. Grieve, and P. B. Robertson. 1988. “Microscopic Lamellar Deformation Features in Quartz: Discriminative Characteristics of Shock-Generated Varieties.” Geology 16 (9): 796–799.
Alvarez, L. W., W. Alvarez, F. Asaro, and H. V. Michel. 1980. “Extraterrestrial Cause for the Cretaceous-Tertiary Extinction.” Science 208 (4448): 1095–1108.
Barboza-Gudino, J. R., R. S. Molina-Garza, and T. F. Lawton. 2012. “Sierra de Catorce: Remnants of the Ancient Western Equatorial Margin of Pangea in Central Mexico.” In The Southern Cordillera and Beyond Field Guide. Edited by J. J. Aranda-Gómez, G. Tolson, and R. S. Molina-Garza, 1–18. Boulder, Colorado: Geological Society of America.
Boslough, M. B. 1989. “A Thermochemical Model for Shock-Induced Chemical Reactions in Porous Solids: Analogs and Contrasts to Detonation.” Proceedings of the 9th Symposium on Detonation, 1199–1209. Silver Spring, Maryland: Naval Surface Warfare Center.
Boslough, M., M. Aftosmis, M. J. Berger, S. M. Ezzedine, G. Gisler, B. Jennings, V. V. Titov, et al. 2016. “Asteroid-Generated Tsunami and Impact Risk.” Abstract NH13A-1763. San Francisco, California: American Geophysical Union Fall Meeting.
Bourgeois, J., T. A. Hansen, P. L. Wiberg, and E. G. Kauffman. 1988. “A Tsunami Deposit at the Cretaceous-Tertiary Boundary in Texas.” Science 141 (4865): 567–570.
Carter, N. L., C. B. Officer, C. A. Chesner, and W. I. Rose. 1986. “Dynamic Deformation of Volcanic Ejecta From the Toba Caldera: Possible Relevance to Cretaceous/Tertiary Boundary Phenomena.” Geology 14 (5): 380–383.
Charig, A. J. 1993. “Disaster Theories of Dinosaur Extinction.” Modern Geology 18: 299–318.
Christeson, G. L., Y. Nakamura, R. T. Buffler, J. Morgan, and M. Warner. 2001. “Deep Crustal Structure of the Chicxulub Impact Crater.” Journal of Geophysical Research 106 (B10): 21751–21769.
Claeys, P., W. Kiessling, and W. Alvarez. 2002. “Distribution of Chicxulub Ejecta at the Cretaceous-Tertiary Boundary.” In Catastrophic Events and Mass Extinctions: Impacts and Beyond. Edited by C. Koeberl and K. G. MacLeod, 55–68, Boulder, Colorado: Geological Society of America Special Paper 356.
Clarey, T. 2015. “The Whopper Sand.” Acts & Facts 44 (3). Dallas, Texas: Institute for Creation Research.
Clemens, W. A., and J. H. Hartman. 2014. “From Tyrannosaurus rex to Asteroid Impact: Early Studies (1901–1980) of the Hell Creek Formation in its Type Area.” In Through the End of the Cretaceous in the Type Locality of the Hell Creek Formation in Montana and Adjacent Areas. Edited by G. P. Wilson, W. A. Clemens, J. R. Horner, and J. H. Hartman, 1–87. Boulder, Colorado: Geological Society of America Special Paper 503.
Cohen, K. M, S. C. Finney, P. L. Gibbard, and J.-X. Fan. 2013. “The ICS International Chronostratigraphic Chart.” Episodes 36 (3): 199–204.
Connors, M., A. R. Hildebrand, M. Pilkington, C. Ortiz-Aleman, R. E. Chavez, J. Urrutia-Fucugauchi, E. Graniel-Castro, A. Camara-Zi, J. Vasquez, and J. F. Halpenny. 1996. “Yucatán Karst Features and the Size of Chicxulub Crater.” Geophysical Journal International 127 (3): F11–F14.
Crocket, J. H., C. B. Officer, F. C. Wezel, and G. D. Johnson. 1988. “Distribution of Noble Metals Across the Cretaceous/Tertiary Boundary at Gubbio, Italy: Iridium Variation as a Constraint on the Duration and Nature of Cretaceous/Tertiary Events.” Geology 16 (1): 77–80.
Ende, M., S. Schorr, G. Kloess, A. Franz, and M. Tovar. 2012. “Shocked Quartz in Sahara Fulgurite.” European Journal of Mineralogy 24 (3): 499–507.
Ferrière, L., and G. R. Osinski. 2013. “Shock Metamorphism.” In Impact Cratering: Processes and Products. 1st ed. Edited by G. R. Osinski, and E. Pierazzo, 106–124. Oxford, England: Blackwell Publishing.
French, B. M., and C. Koeberl. 2010. “The Convincing Identification of Terrestrial Meteorite Impact Structures: What Works, What Doesn’t, and Why.” Earth Science Reviews 98 (1–2): 123–170.
Gale, A. S. 2006. “The Cretaceous-Palaeogene Boundary on the Brazos River, Falls County, Texas: Is There Evidence For Impact-Induced Tsunami Sedimentation?” Proceedings of the Geologist’s Association 117 (2): 173–185.
Gilli, A., D. A. Hodell, G. D. Kamenov, and M. Brenner. 2009. “Geological and Archaeological Implications of Strontium Isotope Analysis of Exposed Bedrock in the Chicxulub Crater Basin, Northwestern Yucatán, Mexico.” Geology 37 (8): 723–726.
Grajales-Nishimura, J., E. Cedillo-Pardo, C. Rosales-Domínguez, D. J. Morán-Zenteno, W. Alvarez, P. Claeys, J. Ruíz-Morales, J. García-Hernández, P. Padilla-Avila, and A. Sánchez-Ríos. 2000. “Chicxulub Impact: The Origin of Reservoir and Seal Facies in the Southeastern Mexico Oil Fields.” Geology 28 (4): 307–310.
Grieve, R. A. F., F. Langenhorst, and D. Stöffler. 1996. “Shock Metamorphism of Quartz in Nature and Experiment: II. Significance in Geoscience.” Meteoritics & Planetary Science 31 (1): 6–35.
Grieve, R. A. F., and M. Pilkington. 1996. “The Signature of Terrestrial Impacts.” AGSO Journal of Australian Geology & Geophysics 16 (4): 399–420.
Gucsik, A. 2009. “Shock Metamorphism of Terrestrial Impact Structures and its Application in the Earth and Planetary Sciences.” In Cathodoluminescence and its Application in the Planetary Sciences. Edited by A. Gucsik, 23–43. Berlin, Germany: Springer-Verlag.
Gulick, S. P. S., G. L. Christeson, P. J. Barton, R. A. F. Grieve, J. V. Morgan, and J. Urritia-Fucugauchi. 2013. “Geophysical Characterization of the Chicxulub Impact Crater.” Reviews of Geophysics 51 (1): 31–52.
Guzmán, E. J., and U.F. Mina. 1953. “Developments in Mexico in 1952.” Bulletin of the American Association of Petroleum Geologists 37 (6): 1506–1522.
Hayes, J. D., J. Imbrie, and N. J. Shackleton. 1976. “Variations in the Earth’s Orbit: Pacemaker of the Ice Ages.” Science 194 (4270): 1121–1132.
Hebert, J. 2014. “Circular Reasoning in the Dating of Deep Seafloor Sediments and Ice Cores: The Orbital Tuning Method.” Answers Research Journal 7: 297–309.
Hebert, J. 2016a. “Revisiting an Iconic Argument for Milankovitch Climate Forcing: Should the ‘Pacemaker of the Ice Ages’ Paper be Retracted?—Part 1.” Answers Research Journal 9: 25–56.
Hebert, J. 2016b. “Revisiting an Iconic Argument for Milankovitch Climate Forcing: Should the ‘Pacemaker of the Ice Ages’ Paper be Retracted?—Part 2.” Answers Research Journal 9: 131–147.
Hebert, J. 2016c. “Revisiting an Iconic Argument for Milankovitch Climate Forcing: Should the ‘Pacemaker of the Ice Ages’ Paper be Retracted?—Part 3.” Answers Research Journal 9: 229–255.
Hildebrand, A. R., G. T. Penfield, D. A. Kring, M. Pilkington, A. Camargo, S. B. Jacobsen, and W. V. Boynton. 1991. “Chicxulub Crater: A Possible Cretaceous/Tertiary Boundary Impact Site on the Yucatán Peninsula, Mexico.” Geology 19 (9): 867–871.
Huffman, A. R., and W. U. Reimold. 1996. “Experimental Constraints on Shock-Induced Microstructures in Naturally Deformed Silicates.” Tectonophysics 256 (1–4): 165–217.
Hurlbut, C. S. Jr., and C. Klein. 1977. Manual of Mineralogy. 19th ed. New York, New York: John Wiley & Sons.
Keller, G., T. Adatte, W. Stinnesbeck, M. Rebolledo-Vieyra, J. U. Fucugauchi, U. Kramar, and D. Stüben. 2004a. “Chicxulub Impact Predates the K-T Boundary Mass Extinction.” Proceedings of the National Academy of Sciences 101 (11): 3753–3758.
Keller, G., T. Adatte, W. Stinnesbeck, D. Stüben, Z. Berner, U. Kramar, and M. Harting. 2004b. “More Evidence that the Chicxulub Impact Predates the K/T Mass Extinction.” Meteoritics & Planetary Science 39 (7): 1127–1144.
Keller, G., and T. Adatte. 2007. “Age and Paleoenvironment of Deccan Volcanism and the K-T Mass Extinction.” Geological Society of America Annual Meeting Abstracts with Programs 39 (6): 566.
Keller, G., T. Adatte, Z. Berner, M. Harting, G. Baum, M. Prauss, A. Tantawy, and D. Stueben. 2007. “Chicxulub Impact Predates K-T Boundary: New Evidence from Brazos, Texas.” Earth and Planetary Science Letters 255 (3–4): 339–356.
Kenkmann, T., A. Wittmann, D. Scherler, and R. T. Schmitt. 2003. “Deformation Features of the Cretaceous Units of the ICDP-Chicxulub Drill Core Yax-1.” Geophysical Research Abstracts 5(05098). European Geophysical Society.
Koeberl, C. 1993. “Chicxulub Crater, Yucatan: Tektites, Impact Glasses, and the Geochemistry of Target Rocks and Breccias.” Geology 21 (3): 211–214.
Koeberl, C., and W. U. Reimold. 1999. “Argument Supporting Explosive Igneous Activity for the Origin of ‘Cryptoexplosion’ Structures in the Midcontinent, Unites States: Comments and Reply.” Geology 27 (3): 281–282.
Kring, D. A., and W. V. Boynton. 1992. “Petrogenesis of an Augite-Bearing Melt Rock in the Chicxulub Structure and Its Relationship to K/T Impact Spherules in Haiti.” Nature 358 (6382): 141–144.
Kring, D. A., F. Hörz, L. Zurcher, and J. Urrutia Fucugauchi. 2004. “Impact Lithologies and Their Emplacement in the Chicxulub Impact Crater: Initial Results From the Chicxulub Scientific Drilling Project, Yaxcopoil, Mexico.” Meteoritics & Planetary Science 39 (6): 879–897.
Kring, D. A. 2007. “The Chicxulub Impact Event and Its Environmental Consequences at the Cretaceous–Tertiary Boundary.” Palaeogeography, Palaeoclimatology, Palaeoecology 255 (1–2): 4–21.
Krogh, T. E., S. L. Kamo, V. L. Sharpton, L. E. Marin, and A. R. Hildebrand. 1993. “U-Pb Ages of Single Shocked Zircons Linking Distal K/T Ejecta to the Chicxulub Crater.” Nature 366 (6457): 731–734.
Kuiper, K. F., A. Deino, F. J. Hilgen, W. Krijgsman, P. R. Renne, and J. R. Wijbrans. 2008. “Synchronizing Rock Clocks of Earth History.” Science 320 (5875): 500–504.
López-Ramos, E. 1975. “Geological Summary of the Yucatán Peninsula.” In The Ocean Basins and Margins. Volume 3: The Gulf of Mexico and the Caribbean. Edited by A. E. M. Nairn and F. G. Stehli, 257–282. New York, New York: Plenum Press.
Lounejeva, E., M. Ostroumov, and G. Sánchez-Rubio. 2002. “Micro-Raman and Optical Identification of Coesite in Suevite from Chicxulub.” In Catastrophic Events and Mass Extinctions: Impacts and Beyond. Edited by C. Koeberl and K. G. MacLeod, 47–54. Boulder, Colorado: Geological Society of America Special Paper 356.
Lyons, J. B., C. B. Officer, P. E. Borella, and R. Lahodynsky. 1993. “Planar Lamellar Substructures in Quartz.” Earth and Planetary Science Letters 119 (3): 431–440.
Martini, M., L. Ferrari, M. López-Martínez, M. Cerca-Martínez, V. A. Valencia, and L. Serrano-Durán. 2009. “Cretaceous-Eocene Magmatism and Laramide Deformation in Southwestern Mexico: No Role for Terrane Accretion.” In Backbone of the Americas. Shallow Subduction, Plateau Uplift, and Ridge and Terrane Collision. Edited by S. M. Kay, V. A. Ramos, and W. R. Dickinson, 151–182. Boulder, Colorado: Geological Society of America Memoir 204.
Melosh, H. J. 1989. Impact Cratering: A Geologic Process. New York, New York: Oxford University Press.
Morgan, J., M. Warner, J. Brittan, R. Buffler, A. Camargo, G. Christeson, P. Denton, et al. 1997. “Size and Morphology of the Chicxulub Impact Crater.” Nature 390 (6659): 472–476.
Morgan, J. V., M. R. Warner, G. S. Collins, H. J. Melosh, and G. L. Christeson. 2000. “Peak-Ring Formation in Large Impact Craters: Geophysical Constraints from Chicxulub.” Earth and Planetary Science Letters 183 (3–4): 347–354.
Morgan, J. V., S. P. S. Gulick, T. Bralower, E. Chenot, G. Christeson, P. Claeys, C. Cockell, et al. 2016. “The Formation of Peak Rings in Large Impact Craters.” Science 354 (6314): 878–882.
Oard, M. J. 2009. “How Many Impact Craters Should There Be On the Earth?” Journal of Creation 23 (3): 61–69.
Oard, M. J. 2012. “An Impact Flood Submodel–Dealing With Issues.” Journal of Creation 26 (2): 73–81.
Oard, M. J. 2013. “What Do Impacts Accomplish in the First Hour?” Journal of Creation 27 (1): 90–98.
Oard, M. J. 2014. “Precambrian impacts and the Genesis Flood.” Journal of Creation 28(3): 99–105.
Paquay, F. S., G. E. Ravizza, T. K. Dalai, and B. Peucker-Ehrenbrink. 2008. “Determining Chondritic Impactor Size from the Marine Osmium Isotope Record.” Science 320 (5873): 214–218.
Pardo, G. 2009. The Geology of Cuba: AAPG Studies in Geology #58. Tulsa, Oklahoma: The American Association of Petroleum Geologists.
Penfield, G. T., and Z. A. Camargo. 1981. “Definition of a Major Igneous Zone in the Central Yucatán Platform with Aeromagnetics and Gravity.” In Technical Program, Abstracts and Bibliographies, Society of Exploration Geophysicists, 51st Annual Meeting, 37. Tulsa, Oklahoma: Society of Exploration Geophysicists.
Peterson, J. A. 1983. “Petroleum Geology and Resources of Southeastern Mexico, Northern Guatemala, and Belize.” Geological Survey Circular 760. Alexandria, Virginia: USGS.
Pope, K. O., S. W. Kieffer, and D. E. Ames. 2004. “Empirical and theoretical comparisons of the Chicxulub and Sudbury impact structures.” Meteoritics & Planetary Science 39 (1): 97–116.
Rabie, R. L., G. R. Fowles, and W. Fickett. 1979. “The Polymorphic Detonation.” The Physics of Fluids 22 (3): 422–435.
Ramsay, J. G., and M. I. Huber. 1983. The Techniques of Modern Structural Geology. Volume 1: Strain Analysis. London, England: Academic Press, Inc.
Rice, A. 1985. “The Mechanism of the Mt. St. Helens Eruption and Speculations Regarding Soret Effects in Planetary dynamics.” Geophysical Surveys 7 (4): 303–384.
Rice, A. 1987. “Shocked Minerals at the K/T Boundary: Explosive Volcanism as a Source.” Physics of the Earth and Planetary Interiors 48 (1–2): 167–174.
Richards, M. A., W. Alvarez, S. Self, L. Karlstrom, P. R. Renne, M. Manga, C. J. Sprain, J. Smit, L. Vanderkluysen, and S. A. Gibson. 2015. “Triggering of the Largest Deccan Eruptions by the Chicxulub Impact.” Geological Society of America Bulletin 127 (11–12): 1507–1520.
Rocchia, R., D. Boclet, P. Bonté, C. Jéhanno, Y. Chen, V. Courtillot, C. Mary, and F. Wezel. 1990. “The Cretaceous-Tertiary Boundary at Gubbio Revisited: Vertical Extent of the Ir Anomaly.” Earth and Planetary Science Letters 99 (3): 206–219.
Rosen, J. 2016. “Crystal clocks.” Science 354 (6314): 822–825.
Saikia, B., G. Parthasarathy, and R. R. Borah. 2015. “Distribution of Microcrystalline Quartz in Glassy Fulgurites from Garuamukh and Kimin, India.” Journal of Applied Mathematics and Physics 3: 1343–1351.
Sarjeant, W. A. S., and P. J. Currie. 2001. “The ‘Great Extinction’ that never Happened: The Demise of the Dinosaurs Considered.” Canadian Journal of Earth Sciences 38 (2): 239–247.
Schulte, P., R. P. Speijer, H. Mai, and A. Kontny. 2006. “The Cretaceous-Paleogene (K-P) Boundary at Brazos, Texas: Sequence Stratigraphy, Depositional Events and the Chicxulub Impact.” Sedimentary Geology 184 (2): 77–109.
Schuraytz, B. C., D. J. Lindstrom, L. E. Marin, R. R. Martinez, D. W. Mittlefehldt, V. L. Sharpton, and S. J. Wentworth. 1996. “Iridium Metal in Chicxulub Impact Melt: Forensic Chemistry on the K-T Smoking Gun.” Science 271 (5255): 1573–1576.
Sharpton, V. L., G. B. Dalrymple, L. E. Marin, G. Ryder, B. C. Schuraytz, and J. Urrutia-Fucugauchi. 1992. “New Links Between the Chicxulub Impact Structure and the Cretaceous/Tertiary Boundary.” Nature 359 (6398): 819–821.
Sharpton, V. L., K. Burke, A. Camargo-Zanoguera, S. A. Hall, D. S. Lee, L. E. Marín, G. Suáarez-Reynoso, J. M. Quezada-Muñeton, P. D. Spudis, and J. Urrutia-Fucugauchi. 1993. “Chicxulub Multiring Impact Basin: Size and Other Characteristics Derived from Gravity Analysis.” Science 261 (5128): 1564–1567.
Sharpton, V. L., L. E Marin, J. L. Carney, S. Lee, G. Ryder, B. C. Schuraytz, P. Sikora, and P. D. Spudis. 1996. “A Model of the Chicxulub Impact Basin Based on Evaluation of Geophysical Data, Well Logs, and Drill Core Samples.” In The Cretaceous-Tertiary Event and Other Catastrophes in Earth History. Edited by G. Ryder, D. Fastovsky, and S. Gartner, 55–74. Boulder, Colorado: Geological Society of America Special Paper 307.
Smit, J., T. B. Roep, W. Alvarez, A. Montanari, P. Claeys, J. M. Grajales-Nishimura, and J. Bermudez. 1996. “Coarse-Grained Clastic Sandstone Complex at the K-T Boundary Around the Gulf of Mexico: Deposition by Tsunami Waves Induced by the Chicxulub Impact.” Geological Society of America Special Paper 307: 151–182.
Smit, J. 1999. “The Global Stratigraphy of the Cretaceous-Tertiary Boundary Impact Ejecta.” Annual Reviews of Earth and Planetary Sciences 27: 75–113.
Smit, J., B. Dressler, R. Buffler, D. Moran-Zenteno, V. L. Sharpton, D. Stöffler, J. Urrutia, and J. Morgan. 2002. “Yaxcopoil-1 Drillhole in the Chicxulub Impact Crater.” Geological Society of America Abstracts with Programs 34 (6): 317.
Snelling, A. A. 2009. Earth’s Catastrophic Past: Geology, Creation & the Flood. Vol. 2, 487–530. Dallas, Texas: Institute for Creation Research.
Snelling, A. A. 2014. “Geological Issues.” In Grappling with the Chronology of the Genesis Flood: Navigating the Flow of Time in Biblical Narrative. Edited by S. W. Boyd and A. A. Snelling, 77–110. Green Forest, Arkansas: Master Books.
Spencer, W. R. 1998a. “Catastrophic Impact Bombardment Surrounding the Genesis Flood.” In Proceedings of the Fourth International Conference on Creationism. Edited by R. E. Walsh, 553–566. Pittsburgh, Pennsylvania: Creation Science Fellowship.
Spencer, W. R. 1998b. “Geophysical Effects of Impacts During the Genesis Flood.” In Proceedings of the Fourth International Conference on Creationism. Edited by R. E. Walsh, 567–579. Pittsburgh, Pennsylvania: Creation Science Fellowship.
Spencer, W. R. 2013. “Impacts and Noah’s Flood—How Many and Other Issues.” Journal of Creation 27 (1): 85–89.
Spencer, W. R. 2014. “Evaluating The Day Four Cratering Hypothesis.” Answers Research Journal 7: 323–329.
Spencer, W. 2015. “ Magnetized Moon Rocks, Impacts, and the Precambrian—A Response to Humphreys.” Journal of Creation 29 (1): 116–119.
Spray, J. G. 1995. “Pseudotachylyte Controversy: Fact or Friction?” Geology 23 (12): 1119–1122.
Stinnesbeck, W., G. Keller, T. Adatte, M. Harting, D. Stüben, G. Istrate, and U. Kramar. 2004. “Yaxcopoil-1 and the Chicxulub Impact.” International Journal of Earth Sciences 93 (6): 1042–1065.
Stöffler, D. 1971. “Coesite and Stishovite in Shocked Crystalline Rocks.” Journal of Geophysical Research 76 (23): 5474–5488.
Tan, Y. J., M. Tolstoy, F. Waldhauser, and W. S. D. Wilcock. 2016. “Dynamics of a Seafloor-Spreading Episode at the East Pacific Rise.” Nature 540 (7632): 261–265.
Taylor, P. F. 2007. “Dinosaur Killer” https://answersingenesis.org/dinosaurs/extinction/dinosaur-killer/.
Telford, W. M., L. P. Geldart, and R. E. Sheriff. 1990. Applied Geophysics. 2nd ed. Cambridge, England: Cambridge University Press.
Tuchscherer, M. G., W. U. Reimold, C. Koeberl, R. L. Gibson, and D. de Bruin. 2004. “First Petrographic Results on impactites from the Yaxcopoil-1 Borehole, Chicxulub Structure, Mexico.” Meteoritics & Planetary Science 39 (6): 899–930.
Ward, W. C., G. Keller, W. Stinnesbeck, and T. Adatte. 1995. “Yucatán Subsurface Stratigraphy: Implications and Constraints for the Chicxulub Impact.” Geology 23: 873–876.
Wittmann, A., T. Kenkmann, R. T. Schmitt, and D. Stöffler. 2003. “Clastic Polymict Dikes in the “Megablock” Sequence of the ICDP-Chicxulub Drill Core Yax-1.” Geophysical Research Abstracts 5 (05223). European Geophysical Society.
Zürcher, L., and D. A. Kring. 2004. “Hydrothermal Alteration in the Core of the Yaxcopoil-1 Borehole, Chicxulub Impact Structure, Mexico.” Meteoritics & Planetary Science 39 (7): 1199–1221.